Research
Advancing Quantum Information Science with Scalable Materials

Spectroscopy and Structural Studies of Colloidal Nanocrystals.
We investigate how structural features, such as crystal symmetry, surface chemistry, and lattice dynamics which govern the optical behavior of colloidal nanocrystals. By combining steady-state and ultrafast spectroscopic techniques, we aim to uncover the mechanisms behind complex phenomena like phonon-assisted up-conversion, exciton-polaron formation, and coherent lattice motion. These insights guide the rational design of nanomaterials for applications in optoelectronics and photonics.

Synthesis and Properties of Nanoparticles
The ability to make nanocrystals of high quality (uniform size, no defects except the ones we want, designed surface, etc.) is key to this area of science, and also interesting in its own right. We grow nanocrystals with well-controlled sizes and shapes by injecting molecular precursors into hot liquids that also contain molecular species that will coordinate with the growing nanoparticle surfaces. Some important questions of solid-state chemistry can be addressed in the synthesis of nanocrystals. How does the nucleation of a solid occur? What governs the rate of growth of each facet of a crystal? What is the stress and strain at the interface between a core and a shell of different materials?
We can think of a simple nanocrystal as a type of artificial atom. In that case, the next step in nanocrystal synthesis is to learn how to make artificial molecules, in which nanocrystals are of differing composition, size, and shape, and interconnected in a designed arrangement. To accomplish this, we develop modular approaches to the chemical transformation of nanocrystals. We are working to understand better the mechanisms of each of nanoscale transformations, as well as searching for new chemical transformations of nanoparticles.
We care about fundamental science of nanocrystals, we are interested in developing automated, self-correcting nanoparticle syntheses, surface derivitization, and methods for nanocrystal characterization and assembly. A feature of our current work is the development of new methods to observe the growth trajectories and formation mechanisms of individual colloidal nanocrystals in liquid solutions.
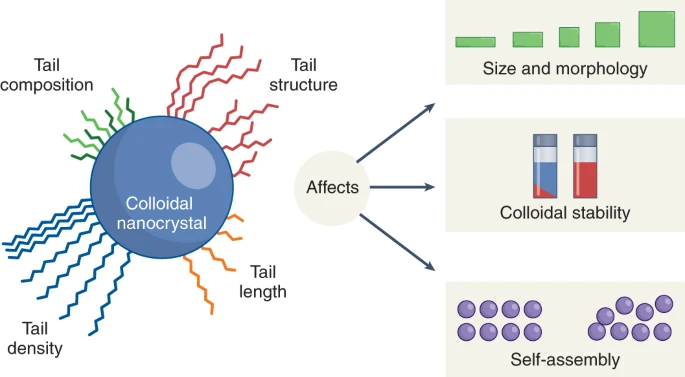

Development of Group III-V Semiconductor Nanocrystals
Our group is dedicated to researching III-V group semiconductor nanocrystals by exploring a variety of synthetic methods that enable precise control over composition, morphology, and size. In doing so, we can attain tunable optical and electronic properties that can be customized for diverse applications.
Compared to traditional semiconductor materials, nanocrystals offer superior abilities and performance for next-generation high-efficiency solar cells, light-emitting diodes, and sensors.
Quantum dots’ unique morphology makes them excellent candidates for optimization utilizing colloidal synthesis techniques. Alteration of the size and type of the surface ligands incorporated into the nanocrystal structure allows for the fine-tuning of optoelectronic properties such as charge transport and quantum yield. The nanocrystals can be synthesized with different ligands or the ligands can be exchanged post-synthesis. We additionally investigate how we can synthesize nanocrystals into different crystal structures via multi-step syntheses involving templating and cation/ anion exchanges, giving us access to crystal structures that are otherwise not possible from direct synthesis.
In order to observe nanocrystal properties such as size, shape, structure, and optoelectronic properties. The knowledge acquired from these investigations will lay the foundation for a new era of eco-friendly, high-performance, and fundamentally interesting materials, revolutionizing industries such as telecommunications, displays, renewable energy, and even art!

Machine Learning Driving Nanomaterials Research
Our group is working on the use of machine learning (ML) to revolutionize the study of nanomaterials, focusing on both the synthesis and characterization of nanostructures. By leveraging automated high-throughput experimentation, we have developed multiple algorithms streamline the analysis of optical spectra and TEM images to extract quantitative information about nanoparticle size, shape, and morphology. These ML models enable the prediction of synthesis outcomes and reaction pathways, significantly accelerating material discovery. Additionally, we employ advanced artificial intelligence techniques such as variational autoencoders to deconvolute complex in-situ TEM datasets, capturing nanoscale dynamics with unprecedented detail. This combination of machine learning and experimental automation is pushing the boundaries of nanomaterial research, allowing us to better understand and control the synthesis of functional nanomaterials for a variety of applications.
Art with Nanoparticles
Craftsmen were arguably the first nanoscientists. Artifacts from as early as the Bronze Age reveal that artisans harnessed nanoscale phenomena to produce a variety of effects: lustre in early Islamic ceramics, enhanced strength in 9th century Syrian swords, and rich colors in ancient Roman, Egyptian, Chinese, and medieval European glass. Historians of science tell us that early craftsmen’s practices-gathering information through direct observation, measuring materials to promote reproducible results, and changing one variable at a time-did much to shape the methodology of science. Walk into a contemporary nanoscience lab, and you will find scientists using techniques that derive from ones developed by artists-for example, nanolithography and Lagmuir-Blodgett film-casting.
Thus, it is natural that an interdisciplinary lab such as ours would include an artist. Our artist-in-residence, Kate Nichols, was initially drawn to using nanomaterials because of her interest in structurally colored Morpho butteflies. (Morpho butterflies appear as brilliant blue despite their lack of blue pigmentation. Their blue color is structural rather than chemical, deriving from nanoscale features within their wings.) Once in our lab, Kate was inspired by medieval artisan’s use of plasmonic nanoparticles in stained glass windows. She synthesizes similar plasmonic nanoparticles and uses them to create macroscale art whose structural colors arise from surface plasmon resonance. In her painting, photography, and writing, Kate continues to explore natural photonic structures. Her work can be seen at The Leonardo, a museum of art and science in Salt Lake City, on her website, and at TED.
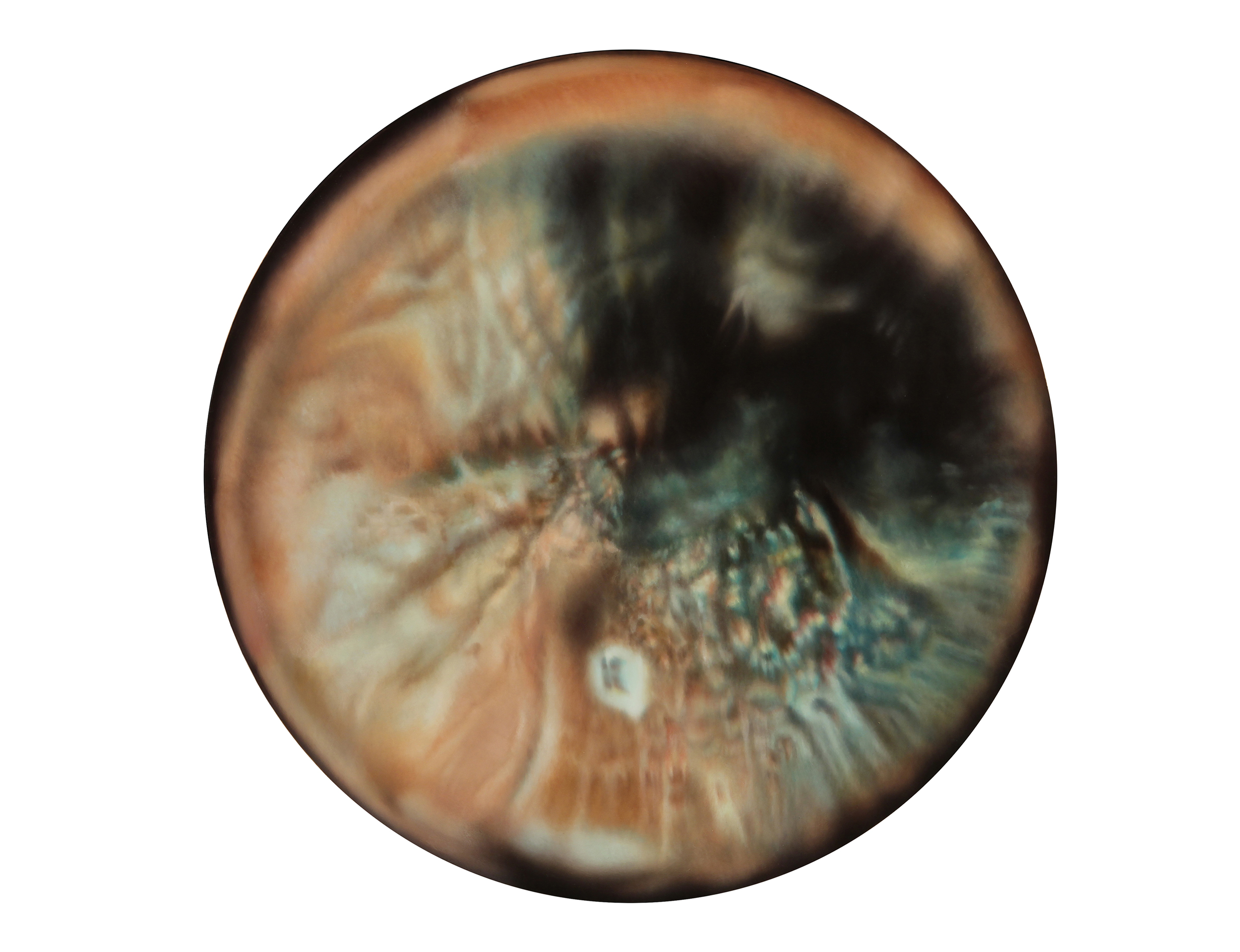
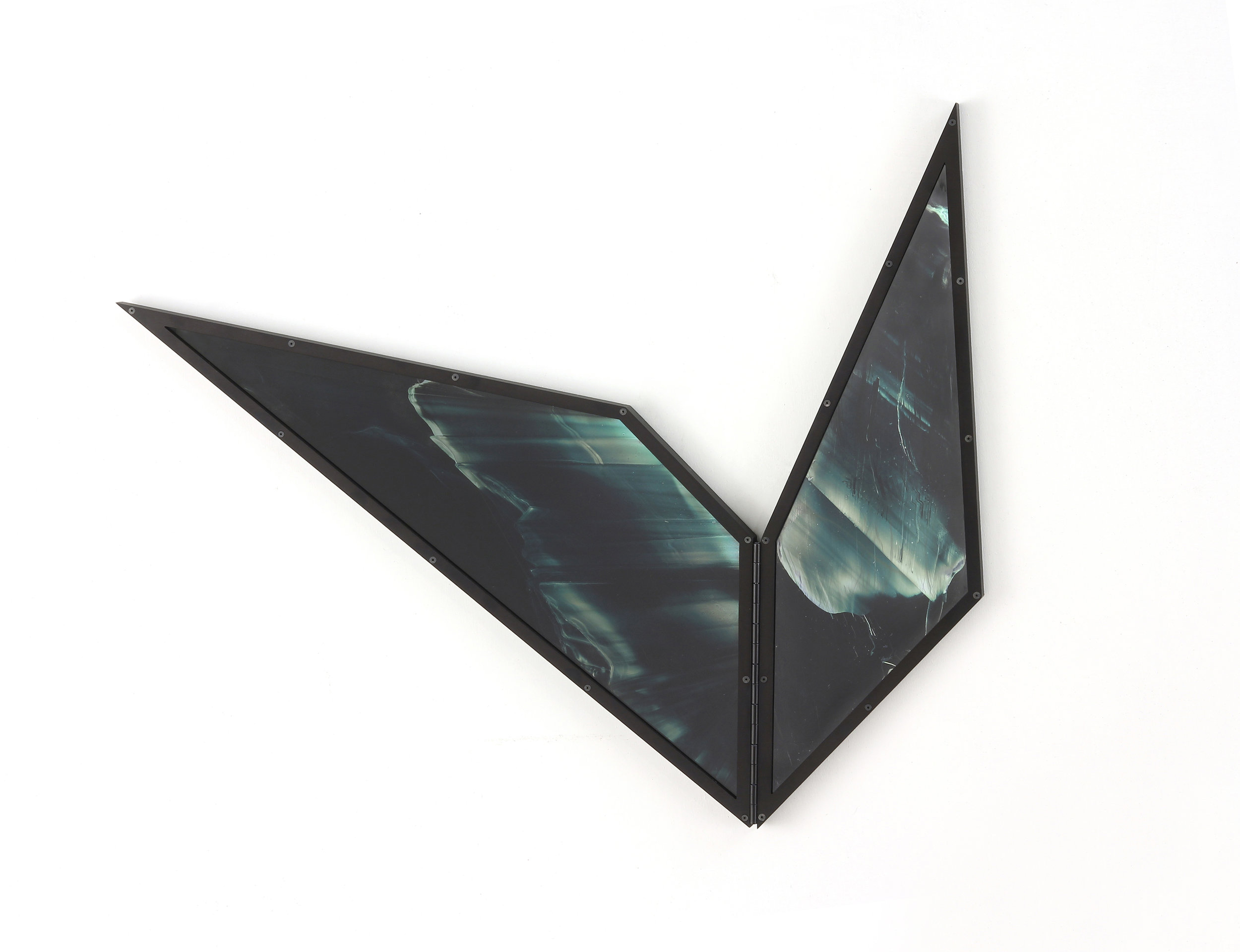